Abstract
Biodegradable polymers are starting to be introduced as raw materials in the food-packaging market. Nevertheless, their price is very high. Starch, a fully biodegradable and bioderived polymer is a very interesting alternative due to its very low price. However, the use of starch as the polymer matrix for the production of rigid food packaging, such as trays, is limited due to its poor mechanical properties, high hidrophilicity and high density. This work presents two strategies to overcome the poor mechanical properties of starch. First, the plasticization of starch with several amounts of glycerol to produce thermoplastic starch (TPS) and second, the production of biocomposites by reinforcing TPS with promising fibers, such as barley straw and grape waste. The mechanical properties obtained are compared with the values predicted by models used in the field of composites; law of mixtures, Kerner-Nielsen and Halpin-Tsai. To evaluate if the materials developed are suitable for the production of food-packaging trays, the TPS-based materials with better mechanical properties were compared with commercial grades of oil-based polymers, polypropylene (PP) and polyethylene-terphthalate (PET), and a biodegradable polymer, polylactic acid (PLA).
Biodegradable; starch; thermoplastic starch; biocomposites; food packaging; natural fibers; barley; grape
Strategies to improve the mechanical properties of starch-based materials: plasticization and natural fibers reinforcement
A. Lopez-GilI; M.A. Rodriguez-PerezII; J.A. De SajaIII
ICellMat Laboratory, Department of Condensed Matter Physics, University of Valladolid, Valladolid, Spain
IIF.S. Bellucci Department of Physics, Chemistry and Biology, São Paulo State University - UNESP, Presidente Prudente, Brasil
IIIM. Ardanuy Department of Textile and Paper Engineering, Universitat Politècnica de Catalunya, Terrassa, Spain
Corresponding author Corresponding author: Alberto López Gil, Cellular Materials Laboratory – CellMat, Condensed Matter Physics Department, Faculty of Sciences, University of Valladolid – UVa, Paseo de Belen, 7, 47011, Valladolid, Spain, e-mail: alopgi@fmc.uva.es
ABSTRACT
Biodegradable polymers are starting to be introduced as raw materials in the food-packaging market. Nevertheless, their price is very high. Starch, a fully biodegradable and bioderived polymer is a very interesting alternative due to its very low price. However, the use of starch as the polymer matrix for the production of rigid food packaging, such as trays, is limited due to its poor mechanical properties, high hidrophilicity and high density. This work presents two strategies to overcome the poor mechanical properties of starch. First, the plasticization of starch with several amounts of glycerol to produce thermoplastic starch (TPS) and second, the production of biocomposites by reinforcing TPS with promising fibers, such as barley straw and grape waste. The mechanical properties obtained are compared with the values predicted by models used in the field of composites; law of mixtures, Kerner-Nielsen and Halpin-Tsai. To evaluate if the materials developed are suitable for the production of food-packaging trays, the TPS-based materials with better mechanical properties were compared with commercial grades of oil-based polymers, polypropylene (PP) and polyethylene-terphthalate (PET), and a biodegradable polymer, polylactic acid (PLA).
Keywords: Biodegradable, starch, thermoplastic starch, biocomposites, food packaging, natural fibers, barley, grape.
Introduction
The packaging market has commonly employed materials such as paper, glass and metals to produce packages for several applications. Nevertheless, these materials have been progressively substituted over the last fifty years by plastic, which is lightweight, resistant to corrosion, presents excellent mechanical properties relative to density, is easy to process by common industrial equipment and last but not least, is cheap. For instance, almost 40% of the plastic consumed worldwide in 2010 belonged to the packaging market [Source: Plastics Europe].
Plastics, in general, are mainly composed of an oil-based polymer matrix mixed with additives such as fillers, plasticizers, lubricants etc., which improve its processability and properties. Such a high diversity in composition makes the recycling of plastics very difficult and the few recycled products obtained are generally of poor quality. For these reasons, plastic waste is usually discarded and accumulated in landfill sites. The high volume of this waste and its slow-rate of degradation, which takes at least 400 years, are dramatically shortening the useful-life of the landfill sites. Several strategies have been set up during the last few years in order to overcome this problem like the incineration and energy recovery of plastic waste and the development of new recycling methods[1]. However, all of them require high investments. Last but not least, petroleum prices have been increased mainly because it is becoming a scarce natural resource, which only few countries possess worldwide. As a result, oil-based resin prices have also been raised.
All these environmental and economic problems are promoting the development of biodegradable polymers whose degradation scarcely takes a few weeks and can be employed for the production of compost[2]. Nevertheless, some of these biopolymers such as PLA, polycaprolactone (PCL) and polyhydroxyalkanoates (PHA) are produced by means of high-cost processes of extraction, fermentation, condensation etc. resulting in very expensive polymers in comparison with oil-based polymers employed in the packaging market such as PP and PET[3-6]. On the contrary, polysaccharides such as starch are directly extracted from cereals, tubers etc. without the need of being synthesized. This fact makes starch even cheaper than oil-based polymers and therefore, an ideal candidate to replace them in applications such as food packaging, a sector that is currently demanding this kind of biodegradable polymers. For all these reasons, the development of a fully biodegradable starch-based material for the production of rigid-food packaging such as trays is currently a challenge not only for scientist but also for industry[7].
Nevertheless, starch also presents several drawbacks, which are preventing the employment of this material for the production of food-packaging trays. These drawbacks are: poor processability in common industrial equipment such as extruders and thermoforming presses, high density (1400 kg/m3), poor mechanical properties after being thermoformed (brittleness) and finally, it is hydrophilic and therefore, wet products cannot be packed.
The rigid packaging market usually employs polymers with high rigidity and strength such as high-density polyethylene HDPE, PP and PET. The high rigidity of these polymers allows the production of trays of low thickness and at the same time, able to support the weight of the food products and other requirements during transport and storage. The low amount of polymer employed for the production of one-single packaging due to its low thickness, results in a drastic reduction of raw-material costs. Nevertheless, native starch by itself is not able to fulfill these mechanical requirements because as was previously mentioned, after being thermoformed, it results in a brittle product, which breaks easily under any loading or impact. Two interesting approaches have been adopted by scientists with the aim of overcoming the poor mechanical properties of starch: first of all, the production of thermoplastic starch (TPS) by the plasticization of native starch with polyols or water[8] and secondly, the production of TPS composites reinforced with natural fibres[9,10]. Several fibres have been traditionally employed such as flax, jute, oil palm to name but a few[11,12]. However, blends of TPS with fibres such as barley straw and grape waste have not been studied in detail yet.
The purpose of this work is the production of materials based on thermoplastic starch able to be employed as the polymer matrix for the production of food-packaging trays. In this sense, two strategies have been developed: on the one hand, the production of TPS with different amounts of glycerol and on the other hand, the production of biocomposites of TPS with natural fibres such as barley straw and grape waste. Models found in literature such as the law of mixtures, Kerner-Nielsen and Halpin-Tsai will be employed in order to evaluate if the fibres are closely adhered and homogeneously orientated and distributed along the TPS matrix and if they fit closely to the experimental results. Finally, the materials produced will be compared, as far as mechanical properties are concerned, with the polymers currently employed in the rigid food-packaging market in order to evaluate if they are suitable to replace the oil-based polymers.
Materials
Wheat starch (Meritena 200) and potato starch were provided by Syral and Tate & Lyle, respectively. Glycerol was supplied by Analar Normapur (99,5% of purity). The barley straw fibers were provided by the department of textiles of the Unversitat Politècnica de Catalunya and the grape waste by Matarromera Group.
Production
Thermoplastic starch is obtained by using plasticizers at high temperatures and shear forces. The high temperatures and shear applied by extruders help the plasticizer to penetrate into the granules of starch and break them resulting in a material with smooth and continuous morphology characteristic of thermoplastics. Apart from morphology, the plasticization of starch also involves changes in its characteristic thermal transitions such as the glass transition temperature, which is shifted from values above 200 °C in the case of native starch to values below 100 °C in the case of TPS. As a result, starch becomes a material, which is easy to process by industrial plastic equipment and with mechanical properties similar to those of oil-based polymers[13].
In this research work a Twin Screw Extruder model Collin Teach Line ZK 25 T SCD 15 was used to plasticize starch and the plasticizer employed was glycerol. The reason for using glycerol was mainly because of its high boiling point (290 °C), which avoided the expansion of TPS by gases at the exit of the extruder. The temperature profile and the screw speed were set to 90-110-130-150-110 °C and 100 rpm respectively. Once thermoplastic starch (TPS) had come out of the die, it was air dried, and later cut into cylindrical pellets. When it comes to the production of biocomposites, the TPS pellets were mixed with natural fibres in a rheometer model Haake 5000. The temperature was set up to 100 °C and the screws speed to 120 rpm. All the materials were thermoformed in a hot-plates press in order to obtain tensile tests specimens. The temperature was set to 150 °C and the pressure applied was 11 MPa.
Three TPS-based formulations with different amounts of glycerol were produced. Wheat Starch (Meritena 200) was employed as the polymer matrix. Table 1 shows these formulations.
Eight biocomposites were produced mixing TPS (30% of glycerol) with different contents of barley straw fibres and grape waste. These formulations are shown in Table 2. In this case, potato starch was employed as the polymer matrix. The reason for using different starches, wheat and potato starch was to study the influence of the botanical origin over the mechanical properties of the final TPS produced.
Characterization
All the materials produced were mechanically and morphologically characterized. A Stereoscopic zoom microscope model SMZ-U Nikon was employed in order to evaluate morphological aspects of the natural fibres such as shape, size and aspect ratio. A Scanning electron microscope model JSM -820 Jeol was employed in order to evaluate the morphology of the TPS samples produced and in the case of the biocomposites, the adhesion degree between the natural fibres and the polymer matrix and its distribution and orientation. Tensile tests were carried out with the aim of evaluating mechanical properties such as rigidity, strength and elasticity. The tests were performed in a Universal testing machine model 5.500R6025 Instron under the standard ISO 37:2011. All the tensile-tests specimens were air-conditioned at the same room conditions (50% of relative humidity) during one week prior to the mechanical tests. The density of all the samples was measured by a gas pycnometer model AccuPyc II 1340, Micromeritics.
Results and Discussion
First of all, the morphology of the TPS-based samples produced with several concentrations of glycerol and natural fibres is shown in the SEM micrographs of the Figure 1a-g, as well as the morphology of both, barley straw fibres and grape waste fibres, respectively shown in the microscopy images of the Figure 1h and i.
As observed in Figure 1 the plasticization process involved the total disappearance of the native starch granules. They were broken by the penetration of the plasticizers, glycerol and water, into the granule with the help of the elevated temperatures and shear applied by the twin-screw extruder. Despite the fact that water was not taken into account when the materials were formulated, starch always presents a certain degree of humidity. The starch water content usually depends on both, the natural origin and the storing conditions. For this reason, glycerol was not the only plasticizer and water also played a key role in this process. The TPS-based materials plasticized with different amounts of glycerol (Figure 1a-c) show a smooth and continuous surface characteristic of thermoplastic materials. In some cases, a small quantity of granules that were not completely broken can be seen. Moreover, some pores are observed, whose number increased when higher amounts of glycerol were employed. The higher the amount of glycerol, the lower the viscosity of the TPS polymer matrix and therefore, water boiling and the formation of pores at the exit of the extruder, is favored by the surrounding polymer[14].
Figure 1h and i show microscopy images of barley straw and grape fibres respectively. The properties of natural fibres, in general, depend on several factors such as the growing conditions, the origin (steam, seed or leaf), structure, chemical composition and morphology[15]. Barley straw is a fibre, which presents higher aspect ratios (25) than the grape waste (2). This fact could have a positive influence on the mechanical properties of the composites produced regarding rigidity and strength. On the contrary, grape waste is a polygonal shaped-natural filler of greater size than the barley straw fibers.
When it comes to the composites only the SEM micrographs of TPS reinforced with 15% of fibres are shown (Figure 1d-g). Both fibres are homogeneously distributed along the TPS matrix and randomly orientated. Nevertheless, interphases between the grape fibers and the polymer matrix are observed in Figure 1g. This could be due to a low degree of adhesion between both materials. On the contrary the barley straw fibres seem to be closely adhered to the TPS matrix as can be seen in the micrograph 1e.
As far as mechanical properties are concerned, tensile tests were carried out in order to evaluate the rigidity, strength and elasticity of all the materials produced. Firstly, the mechanical properties of the TPS plasticized with different amounts of plasticizer are shown in Figure 2.
A decrease in the amount of glycerol from 30% to 20% produced large improvements in the rigidity and strength of the TPS based materials. For instance, the elastic modulus was increased from 16 MPa to 1691 MPa when the amount of glycerol was decreased to 20%. On the contrary, the elasticity was clearly reduced. The starch plasticized with 30% of glycerol presented an elongation at break of 135% whereas in the case of the TPS plasticized with 20% of glycerol it was reduced to 1.2%.
Figure 3 shows the results regarding biocomposites. The addition of barley straw fibres and grape fibres produced very different results, especially in terms of rigidity and strength. For instance, elastic modulus shifted from 90 MPa for the neat TPS matrix to 324 MPa for the TPS reinforced with 15% of barley straw fibres. Tensile strength was also improved and it reached values of 7.2 MPa when adding 15% of barley straw fibres. Nevertheless, the same behaviour was not obtained with the grape fibres. In this case, the rigidity and the strength of the TPS matrix were not significantly enhanced. As for elasticity, the addition of both fibres produced a decrease in the elongation at break. The decrease is lower for the TPS reinforced with grape fibres than for the TPS reinforced with barley straw fibres.
Several facts could explain such a different behavior. On the one hand the morphology and the chemical composition of the fibers. On the other hand the interfacial adhesion, distribution and orientation between the fiber and the polymeric matrix. The aspect ratio was concluded to be possibly playing a key role in the enhanced mechanical properties of the composites reinforced with barley straw fibres. Nevertheless other factors should also be taken into account. The chemical composition, for instance, is very different for both fibres. In the case of the barley straw fibres the cellulose content is 97% while in the case of the grape fibres is 29%.
With regards to the orientation of the fibres, the SEM images do not clarify if they were oriented in a preferential direction. For this reason, a comparison between the experimental results and models found in literature was performed. The model commonly employed for the prediction of the elastic properties of composites is the basic law of mixtures. This law considers that the properties of a composite are between the responses of two basic models: a model in parallel and a model in series, and therefore both phases are under the same strain or stress conditions (Figure 4). The expressions that result for the Young’s modulus are shown in Equations 1 and 2. Equation 1 represents the upper limit or the most optimistic prediction (plane strain) while the bottom limit is defined by Equation 2 (plane stress). Ec, Em and Ep are the Young’s modulus of the composite, polymer matrix (TPS in this case) and the filler (natural fibres in this case). Vp is the filler volumetric fraction.
This model was applied only for the TPS-based biocomposite reinforced with barley straw fibres because of the poor results obtained with the grape fibers. Figure 4c shows the real behaviour of the TPS reinforced with barley straw fibres and the results obtained by the law of mixtures model. The Young’s modulus of the barley straw fibres was calculated taking into account the cellulose and lignin content of this fiber, 96.7% and 1.5% respectively, and typical Young’s modulus values found for them in literature[16].
As shown in Figure 4, both limits are far away from each other and the real behaviour is nearer the bottom than the upper limit and therefore, a plane-stress model is predicted by this law. Nevertheless, because of the long distance between both limits, this model has scarce utility in common composites systems. This model is more suitable for polymer blends than for filler-reinforced polymers.
Kerner developed one of the most widely accepted theories for the prediction of the Young’s modulus of composites reinforced with spherical particles. His model is based on spherical particles dispersed and closely adhered to a polymer matrix. Nielsen adapted this model in order to simplify it and make the application in practical situations easier. Kerner-Nielsen model17 is represented by the Equations 3, 4 and 5.
The Poisson’s coefficient (vm) and the Young’s Modulus (Em) of the polymer matrix and the Young’s modulus (Ep) and the volumetric fraction (Vp) of the particles are defined by the constants A and B.
When it comes to composites reinforced with short fibres, Halpin & Tsai developed a simplified model in order to predict the elastic modulus of unidirectional composites[18]. The Equations 6, 7 and 8 represent this model. The Equation 6 is suitable for composites with the fibres oriented parallel with respect to the stress applied whereas Equation 7 is suitable for the case of the fibres being oriented perpendicularly.
The aspect ratio of the fibres is represented by the parameter ξ. As was shown previously in the microscopic characterization of the natural fibres, the average aspect ratio of the barley straw fibres is 25. Equation 6 is exactly the same as Equation 1 of the law of mixtures and for this reason only Equation 7 for fibres oriented perpendicularly to the stress applied will be represented.
Once all the parameters were introduced for both, Kerner-Nielsen and Halpin-Tsay models, the real and models responses were represented in Figure 5.
As can be seen in Figure 5, the Kerner-Nielsen model did not fit very well with the real behaviour of these biocomposites. On the contrary, the Halpin-Tsai model seems to behave similar to the experimental results. This can be explained taking into account that the Halpin-Tsai model considers the aspect ratio of fibres and the barley straw fibres present an average high aspect ratio of 25. Nevertheless, the Kerner-Nielsen model is more suitable for spherical particles.
Finally, the best results as far as rigidity (Young’s modulus) and strength (Tensile strength) are concerned of the TPS-based materials developed will be compared with those of current polymers employed in the rigid-food packaging market. The oil-based polymers, which were chosen for this comparison, are: HDPE Rigidex HD6070EA (Inneos), PP HB300TF (Borealis) and a common PET found in literature, all of them recommended for rigid packaging applications. Moreover, PLA Ingeo 2003D (NatureWorks) will be also added in order to compare with a bioderived and biodegradable polymer. The mechanical results of all the commercial polymers were obtained from technical data sheets. Figure 6 shows the comparison where the Young’s modulus (a) and the Tensile strength (b) are plotted versus the density of these materials.
As observed in Figure 6a the Young’s modulus of the TPS-based material plasticized with 20% of glycerol (1691 MPa) is even higher than that of the commercial HDPE (1500 MPa) and slightly lower than that of the commercial PP (1700 MPa). However, the TPS-based materials are far from the commercial polymers regarding strength. The Tensile strength of the TPS plasticized with 20% of glycerol is 17.6 MPa and the tensile strength of HDPE is almost double: 31 MPa. Moreover, the density of the TPS-based materials (≈1400Kg/m3) is very high in comparison with the oil-based polymers such as PP (905 Kg/m3), with the exception of PET (1350 Kg/m3) and PLA (1240 Kg/m3). When it comes to the botanical origin of starch (potato or wheat), no significant differences were observed.
Conclusions
This research work describes the production of TPS-based materials with the aim of replacing current oil-based polymers employed in the food-packaging market for the production of trays. The production method employed: extrusion and thermoforming, allowed firstly, plasticizing starch and secondly, the production of biocomposites with enhanced properties in comparison with the neat TPS matrix due to the employment of promising fibres such as barley straw and grape waste. SEM images showed a homogeneous distribution of both fibres along the TPS matrix although interphases between the grape fibres and the TPS matrix were observed, which resulted in poorer mechanical properties than for TPS-barley straw composites. The results of the Halpin-Tsai model were in good agreement with the experimental results of the TPS-based composites reinforced with barley straw fibres. This fact confirmed the importance that the aspect ratio of these fibres had in the final mechanical properties of the composites.
The best result regarding mechanical properties was obtained by reducing the amount of plasticizer up to 20%. This is because the TPS reinforced with natural fibres was plasticized with 30% of glycerol. Although the properties of the TPS-based materials regarding rigidity and strength are in most cases below the properties of the current oil-based polymers, it is fair to point out that the materials developed are completely bioderived, biodegradable and economically competitive, which add an extra value to them. Last but not least, it should be taken into consideration that current policies and laws are promoting the entrance of these biodegradable polymers in the food-packaging market.
Received: 02/06/13
Revised: 10/08/13
Accepted: 01/30/14
- Davis, G. & Song, J. H. - Ind. Crops Prod., 23, p.147 (2006). http://dx.doi.org/10.1016/j.indcrop.2005.05.004
- Kale, G.;
- Ludueña, L.; Vázquez, A. & Alvarez, V. - Carbohydr. Polym., 87, p.411 (2012).
- Auras, R.;
- Corre,
- Chanprateep,
- Mensitieri,
- Jansen, L.
- Satyanarayana,
- Yu, L.; Dean, K. &
- Wollerdorfer, M. &
- Sobczak, L.; Brüggemann,
- BeMiller, J. &
- Li, H.; Michel, A. &
- Kalia, S.; Kaith, B. S.
- Cheng, Q.; Wang, S. &
- Nielsen, L. E. – J.
- Affdl, J. C. H. &
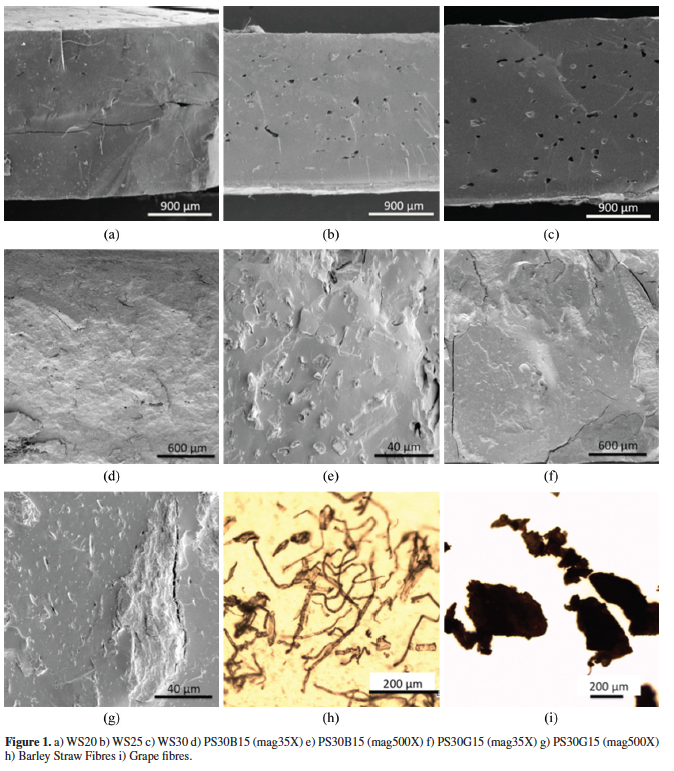
Publication Dates
-
Publication in this collection
20 May 2014 -
Date of issue
2014
History
-
Accepted
30 Jan 2014 -
Received
06 Feb 2013 -
Reviewed
08 Oct 2013