Abstract
The application of a combined system of a polyelectrolyte, carboxymethyl cellulose (CMC), and highly fibrillated fibrous materials, cellulose triacetate fibrets (CTF), for the recovery of proteins and other biological compounds from model and actual biological systems has been demonstrated . In the present work, reaction batches were scaled-up to a one-liter agitated vessel, with a standard configuration. The effect of mixing conditions on the adsorption and flocculation process was studied. It was observed that flocculation time was very fast, occurring within the period of polymer addition. Long term shearing did not result in floc breakage and the values of percentage light transmission and protein concentration of the final filtrate remained the same during the incubation period. Increasing the shear rate resulted in improved process efficiency, up to an optimum value, above which performance was poorer. Perikinetic and orthokinetic rate parameters were calculated and results analyzed in view of these parameters.
Fibrous materials; polyelectrolytes; flocculation; perikinetic; orthokinetic; mixing conditions
EFFECT OF MIXING CONDITIONS ON FLOCCULATION KINETICS OF WASTEWATERS CONTAINING PROTEINS AND OTHER BIOLOGICAL COMPOUNDS USING FIBROUS MATERIALS AND POLYELECTROLYTES
L.A. CHEN1,* * Current address: DuPont White Pigments & Mineral Products, P. O. Drawer 219, New Johnsonville, TN 37134, USA , G.A. SERAD2 and R.G. CARBONELL1** * Current address: DuPont White Pigments & Mineral Products, P. O. Drawer 219, New Johnsonville, TN 37134, USA
1Department of Chemical Engineering, North Carolina State University, Raleigh, NC 27695-7905, USA
2Hoechst Celanese Corporation, P.O. Box 32414, Charlotte, NC 28232-6085, USA
(Received: March 9, 1998; Accepted: October 1, 1998)
Abstract - The application of a combined system of a polyelectrolyte, carboxymethyl cellulose (CMC), and highly fibrillated fibrous materials, cellulose triacetate fibrets (CTF), for the recovery of proteins and other biological compounds from model and actual biological systems has been demonstrated . In the present work, reaction batches were scaled-up to a one-liter agitated vessel, with a standard configuration. The effect of mixing conditions on the adsorption and flocculation process was studied. It was observed that flocculation time was very fast, occurring within the period of polymer addition. Long term shearing did not result in floc breakage and the values of percentage light transmission and protein concentration of the final filtrate remained the same during the incubation period. Increasing the shear rate resulted in improved process efficiency, up to an optimum value, above which performance was poorer. Perikinetic and orthokinetic rate parameters were calculated and results analyzed in view of these parameters.
Keywords: Fibrous materials, polyelectrolytes, flocculation, perikinetic, orthokinetic, mixing conditions.
INTRODUCTION
In the present work, wastewater samples containing proteins, cells and other biological compounds in the colloidal size range are destabilized by a combined system of cellulose-based fibrous material (cellulose triacetate fibrets, CTF) and water-soluble polymer or polyelectrolyte (carboxymethyl cellulose, CMC). The CTF provide a surface for protein adsorption by hydrophobically-driven interactions, as well as a highly fibrillated structure for entrapment of micron-size particles, while the CMC promotes flocculation of the colloidal-size biomolecules and cells by electrostatic interaction and formation of polymer bridges between particles . A better understanding of the various steps of the flocculation process in the case of the CMC+CTF system was achieved. Reaction batches were scaled up to a one-liter agitated vessel with a standard configuration. Reactor conditions -- both mixing intensity and duration -- during the adsorption/flocculation process and the effects that these conditions have on protein concentration and percentage light transmission of the final samples after filtration were considered. Perikinetic and orthokinetic rate parameters were calculated and the results analyzed in view of these parameters.
Ultimately, a mechanistic interpretation of the experimental results of this work can provide information that will aid in the improved design and operation of large-scale reactors for the removal of proteins and other biological compounds from solution using the CMC+CTF process.
THEORETICAL BACKGROUND
Kinetic Aspects of Flocculation
Flocculation of particles in a liquid depends on collisions between particles, caused by their relative motion. This relative motion may be caused by Brownian movement, by fluid movement giving rise to velocity gradients, or by particle motion due to an external force, e.g. gravity .
Brownian or perikinetic flocculation
All colloidal particles undergo a small-scale random motion as a result of their bombardment by molecules of fluid. This Brownian motion is a random, diffusion-type transport, the intensity or rate of which is a function of the thermal energy of the fluid. The rate constant, , for Brownian or perikinetic flocculation is given by
(1)
where is Boltzmann's constant (1.38 x 10-16 erg K-1), T is the absolute temperature (K) and m is the absolute viscosity. This expression was derived from the original theory of diffusion-limited rate constant of doublet formation by Smoluchowski . A detailed derivation can be found elsewhere .
According to equation 1, the collision rate for Brownian transport increases with increasing temperature. A minimum collision rate occurs between particles of identical sizes. For example, given equal number concentrations of 0.01 m m diameter viruses and 1 m m diameter bacteria, the rate of collision between bacteria and viruses is almost thirty times greater than that between viruses or that between bacteria.
Velocity gradient or orthokinetic flocculation
When the relative motion of particles is caused by the bulk motion of the fluid, the term orthokinetic flocculation is used. In the usual mixing units in a flocculation system, the flow is turbulent. Frequency of contact between particles in such flows increases substantially in comparison with the number of encounters in a motionless medium. Orthokinetic flocculation by turbulent fluid motion was considered by Saffman and Turner . They assumed that turbulence is homogeneous and isotropic and that the particles are neutrally buoyant and move with the fluid. The coagulation radius of the particles was assumed to be very small in comparison with the microscale of turbulence, l t. It was also assumed that there is complete containment of colloidal particles within the turbulent eddy and that the mechanism of contact between suspended particles occurs on a scale lower than the size of the eddy. The analysis employed is similar to that used for Brownian flocculation except that instead of relating the diffusional motion of the particles to the thermal energy of the fluid, the kinetic energy of small-scale turbulent fluid motion is used. The rate constant for orthokinetic flocculation caused by small-scale turbulent fluid motion is given by
(2)
Here G is the mean velocity gradient or shear rate (s-1) and is given by
(3)
where e is the rate of energy dissipation by turbulence per unit mass of fluid and n is the kinematic viscosity.
Collision efficiency
The rate constants presented above describe the rate at which collisions between particles occur but not the rate at which lasting contacts occur. The analyses used in deriving these rate expressions were restricted to single-sphere hydrodynamics and to an attractive potential that is zero for all but touching particles. In order to predict the actual rate of occurrence of lasting contacts, i.e., the proportion of all collisions which lead to aggregation, it is necessary to consider long-range interparticle potential and a rigorous hydrodynamic analysis. In general, hydrodynamic forces and interparticle repulsion reduce the formation of lasting contacts and so it has been customary to express corrections of the simple collision rate, using the collision efficiency factor, a . In the case of the interaction of particles in an orthokinetic encounter, the inclusion of hydrodynamic forces in the analysis has a significant effect on the collision efficiency factor. According to the results of van de Ven and Mason , when double-layer repulsion is negligible, a is approximated by the expression
(4)
where A is the Hamaker constant (10-20J) and r is the radius of the interacting particles in meters. Reduction of the flocculation rate by a factor of 5 has been observed when a was taken into account.
Disaggregation of Flocs
In many practical applications it is necessary to produce strong flocs, which can withstand a high shear. Despite a considerable amount of effort, there is still a lack of fundamental knowledge of the factors which affect the strength of flocs and the nature of floc disaggregation (breakup, erosion, fragmentation, etc.) under various conditions . Most practical interest is in the breakup of flocs under turbulent conditions, where very complex behavior is found . The nature of the breakup process depends on the size of flocs relative to the turbulence microscale, which is typically on the order of 100 m m. For flocs larger than this, deformation and rupture may occur as a result of fluctuating dynamic pressure. For smaller flocs, viscous shear forces predominate and may cause either an erosion of primary particles from the floc surface or fragmentation of the flocs.
It is well known that polymeric flocculation can produce stronger flocs than more conventional agents, presumably because of the formation of polymer bridges between particles with many points of attachment. Estimates of the force required to break a carbon-carbon bond in a polymer chain are in the range of 10-8 to 10-9N , which may be much smaller than the hydrodynamic force between particles in agitated suspensions. A large number of chains would need to be adsorbed on particles in order to produce flocs able to withstand a high shear. When flocs are disrupted by shear, changes in the configuration of the adsorbed chains, as well as chain scission, may occur, so that re-flocculation may not proceed easily when the shear is reduced. It has been suggested that the reversibility of floc breakage under shear may be an indication of whether polymer bridging or charge neutralization is the predominant flocculation mechanism. Charge neutralization generally produces weaker flocs than bridging, but the flocs readily reform.
Initial Mixing -- Turbulent Aspects
The initial mixing unit in a flocculation process is designed to provide close encounters between colloidal particles and flocculant agents. These encounters are controlled by the hydrodynamic parameters, geometry and molecular properties of the solution to be treated and the kinetics of the flocculation reactions . Most of the applications of these flocculation processes are carried out under a turbulent regime . For interpretation of the energy spectrum of such a turbulent hydrodynamic regime, Kolmogoroff's universal equilibrium range theory is generally adopted .
A turbulence or eddy is characterized by its velocity (ul) and scale of turbulence (l t); this is the distance within which the velocity of an eddy changes . The scale of turbulence determines the energy content of an eddy. Large eddies contain the energy of the system. They are responsible for the distribution of the (mixing) energy through the flocculation vessel (kinetic energy and pressure energy). They will transmit their energy to very small eddies which will dissipate the energy of the system. The energy thus cascades from the largest to the smallest eddies. The smallest eddies must be in a state of equilibrium since they are unaffected by boundaries and mean flow. The smallest eddies (microscale of turbulence, l t), which are in a state of equilibrium, must depend only on the rate of energy dissipation, e , and the kinematic viscosity, n . This Theory of Universal Equilibrium was first postulated by Kolmogoroff . The upper zone, the zone in which energy is dissipated by inertion convection, is separated from the viscous energy convection zone by Kolmogoroff's microscale of turbulence (l t).
The dimensions of energy dissipation and kinematic viscosity are e (L2T-3) and n (L2T-1). Using dimensional analysis, the only combination of these parameters which gives a length and a velocity is:
(5)
and
(6)
Thus, eddies which transmit energy and those which dissipate energy are not the same, and they are independent of one another.
Estimate of energy dissipation in a stirred tank
The traditional design approach for flocculation vessels has been to use the velocity gradient or G value to determine the power requirements of the agitator motor using the equation given by
(7)
in which is the mean power input and V is the volume of the vessel.
It is well known that the power required for mixing in fully turbulent tanks is independent of viscosity. For example, for fully baffled cylindrical tanks, the power required is given by
for Re > 10,000 (8)
where
(9)
Here P0 is a constant for turbulent range, depending on impeller details, impeller-tank shape factors and the presence of baffles. P0 is sometimes called the power number, r is the mass density, N is the impeller rotational speed, D is the impeller diameter, and Re is the Reynolds number.
MATERIALS AND METHODS
Materials
Carboxymethyl cellulose (CMC), average MW 250,000, DS 0.7, was purchased from Polysciences, Inc. (Warrington, PA). Citric acid, sodium citrate, hydrochloric acid, sodium hydroxide and miscellaneous chemicals were all of an analytical grade. Cellulose triacetate fibrets (CTF), DS 3.0, were donated by the Hoechst Celanese Corporation (HCC) (Charlotte, NC). Coomassie Blue for the Bradford dye binding assay was from BioRad (Richmond, CA).
Water Sample Collection
Wastewater samples were collected at a commercial poultry processing plant on a weekly basis and transported on ice to the laboratory within two hours. The samples were stored at 5-8° C, and disposed of after one week. Samples of the final effluent from the plant after anaerobic, aerobic and chlorination treatment were also used as standards.
Analysis of Filtrates
The present treatment process was monitored by measuring water clarity and protein concentration of the filtrates and comparing these to the initial values from the raw wastewater samples and to the final values of the effluent from the plant after chlorination (standard). Water clarity of the filtrates and the untreated samples was monitored by absorbance readings at 500 nm using a spectrophotometer (UV160 Shimadzu, Kyoto, Japan) and converted to percentage light transmission, %T . Deionized water, with a specific conductivity of 18 megaohm/cm, was used as a blank. The dissolved protein content of the solutions was monitored by the dye-binding assay . The calibration curve was based on a standard protein, bovine serum albumin (BSA), from Pierce (Rockford, IL). Therefore, the protein concentrations reported here are expressed in equivalent BSA units.
Methods
Flocculation was performed in a baffled, 1000 mL batch reactor at 25° C. The reactor was agitated with a 45° , four-pitched-blade turbine for which power correlations have been developed . Design ratios for the reactor, baffles, and impeller are given in Figure 1. The agitator shaft was driven by a StedFast stirrer, Model SL 600, from Fisher Scientific (Pittsburgh, PA) for speeds up to 720 rpm. For higher speeds, a Virtis Hi-speed 23 Homogenizer (Gardiner, NY) was used. The agitator speed was measured by a 770 digital photoelectric tachometer from TIF Instruments, Inc. (Miami, FL).
Initial pH, protein concentration and percentage light transmission of the wastewater samples were determined, and the pH was then adjusted to 4.0 using 1 M HCl. pH measurements were done using an Accumetâ pH meter 925 from Fisher Scientific (Pittsburgh, PA) with a Rossâ Sure-Flow electrode from ATI Orion (Boston, MA). CMC solution (1 mg/mL) was prepared by dispersing and dissolving the polymer in 0.01 M citrate buffer, with a pH of 4.0, under continuous stirring and heating until it was completely dissolved. A volume of 800 mL wastewater at a pH of 4.0 was mixed with the optimal dosage of CTF [ 1.0 g/g initial protein, as determined by ] for 1 hour, at the appropriate stirring speed, until the fibrets were completely dispersed. The fibrets are supplied as oblong particles of around 2 mm with a high water content. They must be completely dispersed in solution in order to take advantage of their main characteristics of high surface area (20 m2/g) and highly fibrillated structure. Typical sizes of the well-dispersed fibrets in aqueous solution range from 5-60 m m in diameter and 100-600 m m in length, as observed by microscope . Optimal CMC dosage (0.13 g/g protein) was then added from a reservoir above the agitated reactor (Figure 1), while stirring (addition time was around 40 s). The range of impeller speeds studied in this work was from 100 to 900 rpm. The lower limit of the stirring speed was set by tachometer (the lowest accurate measurement was around 100 rpm). The highest speed reached by the StedFast stirrer was 720 rpm. For 900 rpm, the Virtis homogeneizer was used.
Samples of 3 mL were carefully taken from the agitated vessel at predetermined time intervals using a 5 mL disposable pipet. Samples were taken from about the same points in the reactor (close to the impeller) since spatial and directional characteristics near vessel boundaries (agitator and baffle edges, container walls) differ from those far from such boundaries. These samples were filtered in vacuum with 20 m m pore size polyethylene frits (Alltech, Deerfield, IL), using 3 cc disposable syringes [ see for the experimental setup ]. The filtrate was recovered for water clarity and protein analysis as described above. The effects of stirring speed and flocculation time on protein removal and turbidity reduction from the wastewater samples were studied, using CMC and the combined CMC+CTF system.
RESULTS AND DISCUSSION
Using a BSA calibration curve, the initial protein concentration of the wastewater samples was determined to be around 500 mg/L. The density of the slurry was measured by weighing various volumes of the final samples and was calculated to be r = 996 kg/m3 at 25° C, close to that of water. The absolute viscosity was then assumed to be around 1 cP. The root-mean-square fluid velocity gradient or mean shear rate, G, within the reactor was calculated from correlations for a standard tank with a four-pitched-blade impeller using P0, the power number, corrected for the present reactor design . The general assumption of homogeneous, isotropic turbulence of the small eddies, as described in the Theoretical Background section, has been adopted and is an idealization of the actual system.
Figure1
: Reactor configuration and impeller design.
Effect of Stirring Time
Figures 2(a) and (b) show the percentage light transmission and the protein concentration of the final filtrates at various stirring speeds and incubation times for the flocculation using CMC and CMC+CTF. It can be observed from these results that the final values for water clarity and protein concentration do not change significantly with time after addition of CMC: flocculation takes place within the period of polymer addition (40 seconds). CMC alone results in flocculation, but the flocs thus formed are mostly smaller than 20 m m, the pore size of the polyethylene frit used, and remain in the filtrate. CMC+CTF resulted in final filtrates with high water clarity and low protein content, according to previous observations . By comparing the protein concentrations of the untreated samples at pHs of 7.5 (~500 mg/L) and 4.0 (~300 mg/L), it can be noted that about 40% of the proteins in solution are destabilized by the acidic conditions (pH precipitation).
Figure 2: (a) Percentage light transmission and (b) protein concentration of the final filtrate after treatment with CMC only (closed symbols) and CMC+CTF (open symbols) at various stirring speeds: ( ) 100 rpm; (
) 180 rpm; (
) 540 rpm; (
) 720 rpm; (
) 960 rpm. Horizontal solid lines represent the untreated (initial) (X ) and the standard (final) (
) values.
When CTF are added and samples are incubated for 1 hour, the protein concentration goes down to ~200 mg/L, implying that another 20% of the soluble protein is removed from solution by adsorption on the fibrets (hydrophobic interactions), whereas the previously destabilized 40% is removed by physical entrapment within the highly fibrillated microstructure of the CTF. Finally, when CMC is added to the CTF+wastewater suspension, flocculation occurs instantaneously (see below for kinetic calculations), removing the dissolved protein down to a final concentration of ~20 mg/L. By charge-charge interactions between the CMC and the free and CTF-adsorbed proteins, polymer bridges containing adsorbed protein are formed around the fibrets, and the primary particles, formed between CMC and free soluble protein, result in large and strong flocs, which are easily retained by the 20 m m frit. Those smaller flocs that are not attached to any fibret are also retained by entrapment, resulting in a clear filtrate with high water clarity.
Effect of Stirring Speed
Average values of percentage light transmission and protein concentration of the final filtrates from 40 s to 150 minutes of flocculation time were calculated and plotted against stirring speed, N, mean gradient velocity or shear rate, G, and Kolmogoroff's microscale of turbulence, l t, as presented in Figure 3. It can be observed from these plots that an optimum agitation speed exists for this process. Increasing shear has two opposite effects on flocculation: higher collision efficiency leading to aggregate growth and improving floc breakup. The optimum G value is the one below which the kinetics are much worse and above which breakage of flocs predominates . In the case of protein flocculation by polymers, it has been postulated that enhanced dispersion of the polymer at high shear rates allows for its more efficient use, leading to stronger aggregates or improved collision efficiency . Flocculation of clay suspensions by polyelectrolytes showed that flocs ruptured at G values > 350 s-1 . In the present work, the optimum stirring condition was found to be at a shear rate of ~300 s-1, a value also recommended by the Environmental Protection Agency for rapid mixing of municipal wastewater.
Flocculation Kinetics
The appropriate rate equations have been given (equations 1 and 2), and it is possible to make estimates of the different rates. Figure 4(a) shows the perikinetic and orthokinetic rate constants calculated for the range of particle diameters and G values studied. The polymer radius is assumed to be 1000 Å, as given by Rice and Harris . Proteins have sizes around 20 to 100 Å, while cellulose triacetate fibrets range from tens to hundreds of microns. It can be observed from the plot, that for interactions between soluble proteins and CMC, perikinetic flocculation is the controlling rate, independent of the G values in the range of operating conditions studied. On the other hand, for interaction of the polymer with the CTF or protein-adsorbed on the CTF, the orthokinetic rate is controlling.
From the calculated rates, the characteristic flocculation time of the suspension can be determined by
(10)
where is the time in which the number of particles is reduced to half the initial value as a result of aggregate formation (assuming every collision is effective). Alternatively,
can be regarded as the average time in which a particle experiences one collision.
is the number of molecules of component 1 added to the solution.
For the range of protein and polymer concentrations in the samples studied, an estimate of can be obtained and plotted against the range of particle diameters present in the suspension. Figure 4(b) shows the calculated
values for polymer particles of 1000 Å at a concentration of 1.6x1014 molecules/mL [
, calculated from the amount of CMC added to the reactor ]. It can be observed from the plot that flocculation times are on the order of 10-4 to 10-9 seconds for the range of concentrations and particle sizes studied. This explains the experimental results, which showed completed flocculation within the period of polymer addition (40 s). If the collision efficiency, a , is calculated for the range of G values studied here, using equation 4, it can be observed that the flocculation rates would be reduced by a factor of ~3 for the highest stirring speed. This implies that flocculation times will be lowered about three times from those calculated by equation 10. Nevertheless, these corrected flocculation times are still lower than the polymer addition time of 40 seconds.
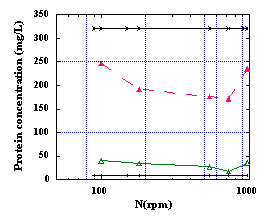
(a)
(b)
(c)
Figure
3: Percentage light transmission and protein concentration of the final filtrate after treatment with CMC (

) and CMC+CTF (D) plotted against (a) stirring speed (
N), (b) mean velocity gradient (
G) and (c) Kolmogoroff's microscale of turbulence. Horizontal solid lines represent the untreated (initial) ( X ) and the standard (final) (

) values.
Since flocculation times are so fast compared to polymer addition time, it is certainly desirable to mix in the polymer as rapidly as possible upon adding it. This would avoid local overdosing and a possible restabilization of a fraction of the particles. If the droplets of added polymer solution are not rapidly dispersed (which may be the case for very viscous solutions), they may act as nuclei for floc formation . On studying flocculation of proteins (lysozyme) by polyelectrolytes (polyacrylic acid), Chen and Berg concluded that the polymer addition process is important in determining the mechanism of floc formation.
(a) Perikinetic and orthokinetic rate constants and (b) characteristic flocculation times for the range of particle diameters and shear rates studied.
The advantage of adding CTF to this flocculation process is also clear from the present results. After flocculation is complete, it seems that flocs formed in the presence of CTF are not easily broken and longer mixing times will not lead to lower protein recoveries. When only CMC is used to promote flocculation of the colloidal particles in the wastewater samples studied, smaller-sized flocs, which seem to be broken at long-term shearing, are formed. This behavior has also been observed by previous studies on the subject .
CONCLUSIONS
The present study confirmed the advantage of the CMC+CTF system over that using CMC. When flocculation of the wastewater samples by addition of CMC is carried out in the presence of CTF, larger flocs that are not easily broken by shearing are formed. These flocs are easily retained by a large-pore-size filter (20 m m), resulting in a dry filter cake and a clear filtrate with low turbidity and low protein concentration. The recovered solids contain proteins and other biological compounds, as well as cellulose-based fibers (CTF) and polymer (CMC) that can be reused as additives for animal feed.
After complete dispersion of the CTF in the samples treated, the flocculation process takes place instantaneously upon addition of the polyelectrolyte. Flocculation times for the range of stirring speeds and particle sizes in the present system are very fast compared to polymer addition times. The effect of shear rate has also been demonstrated, and an optimum mean velocity gradient of 300 s-1 is recommended for this system at the operating conditions studied. The reactor configuration used is based on standard dimensions , and the impeller is a pitched-blade turbine, with inclined blades at 45° angle. Scale-up from these dimensions to an industrial scale should be fairly straightforward.
The present process is not limited by flocculation time, as observed from the experimental results and calculations. It is actually limited by the dispersion of CTF in the sample treated. During dispersion time, some of the soluble proteins adsorb on the fibrets surface. When the polyelectrolyte is added, charge-charge interactions with the free protein molecules in solution, as well as with the adsorbed ones, result in flocculation. Polymer bridges may be formed around the fibrets, the proteins, the primary particles, and the suspended solids, leading to floc growth. The strength of the flocs is such that they are not broken even at long-term shearing or high stirring speeds (within the range studied here). Therefore, a simple filtration process after flocculation results in high recovery of the suspended solids (flocs) and high water clarity of the final filtrate.
ACKNOWLEDGMENTS
The authors wish to thank the Hoechst Celanese Corporation, the William R. Kenan Jr. Institute for Engineering, Technology and Science at NCSU, the Fulbright Commission and the American Chamber of Commerce in Brazil for their financial support during the course of these investigations. Mr. C. Wayne Black, manager of wastewater treatment at a local commercial poultry processing plant is acknowledged for providing the appropriate samples.
REFERENCES
Amirtharajah, A., Initial Mixing. pp. 1-22. In: AWWA Seminar on Coagulation and Filtration: Back to the Basics, St. Louis, MO (1981).
Bradford, M.M., A Rapid and Sensitive Method for the Quantitation of Microgram Quantities of Protein Utilizing the Principle of Protein-Dye Binding. Analyt. Biochem. 72: 248-254 (1976).
Camp, T.R. and Stein, P.C., Velocity Gradients and Internal Work in Fluid Motion. J. Boston Soc. Civ. Engrs. 30: 219 (1943).
Carbonell, R.G.; Chen, L.A. and Serad, G.A., Methods of Treating Wastewater. U.S. Patent No. 5,695,647 (1997).
Chang, Y.H.; Sheldon, B.W. and Carawan, R.E., Research Note: Reconditioning of Prechiller Overflow Water Using a Diatomaceous Earth Pressure Leaf Filter. Poultry Sci. 68: 447-449 (1989).
Chen, L.A., Recovery of Proteins and Other Biological Compounds from Model and Actual Systems Using Fibrous Materials. Ph.D. Dissertation, North Carolina State University, Raleigh, NC (1996).
Chen, L.A., Serad, G.A. and Carbonell, R.G., Protein Recovery Using Fibrous Materials, pp. 83-98. In: Butterfield, D. A. (ed.), Biofunctional Membranes. Plenum Press, New York (1996).
Chen, W. and Berg, J.C., The Effect of Polyelectrolyte Dosage on Floc Formation in Protein Precipitation by Polyelectrolytes. Chem. Eng. Sci. 48(10): 1775-1784 (1993).
EPA, Suspended Solids Removal. Process Design Manual. Environmental Protection Agency, Washington, D.C. (1975).
Fisher, R.R. and Glatz, C.E., Polyelectrolyte Precipitation of Proteins: I. The Effects of Reactor Conditions. Biotech. Bioeng. 32: 777-785 (1987).
François, R.J., Strength of Aluminium Hydroxide Flocs. Water Research. 21(9): 1023-1030 (1987).
Glasgow, L.A. and Luecke, R.H., Mechanisms of Deaggregation for Clay-Polymer Flocs in Turbulent Systems. Ind. Eng. Chem. Fund. 19: 148-156 (1980).
Gregory, J., Kinetic Aspects of Polymer Adsorption and Flocculation, pp. 31-45. In: Attia, Y. A. (ed.), Flocculation in Biotechnology and Separation Systems. Elsevier Science Publishers B. V., Amsterdam (1987).
Hiemenz, P.C., Principles of Colloid and Surface Chemistry, 2d ed. Dekker, New York (1986).
Ives, K.J., Rate Theories, pp. 39-61. In: Ives, K. J. (ed.), The Scientific Basis of Flocculation. Sijthoff & Noordhoff, Alphen aan den Rijn - The Netherlands (1978).
Kolmogoroff, A.N., The Local Structure of Turbulence in Incompressible Viscous Fluid for Very Large Reynolds Numbers. C. R. Acad. Sci. U. S. S. R. 30: 301 (1941).
Kolmogoroff, A.N., On Degeneration of Isotropic Turbulence in an Incompressible Viscous Liquid. C. R. Acad. Sci. U. S. S. R. 31: 538 (1941).
Letterman, R.D., Flocculation. pp. 45-57. In: AWWA Seminar on Coagulation and Filtration: Back to the Basics, St. Louis, MO (1981).
Leu, R.-J. and Ghosh, M.M., Polyelectrolyte Characteristics and Flocculation. J. AWWA. 80(4): 159-167 (1988).
Levich, V.G., Physicochemical Hydrodynamics, 2d ed. Prentice-Hall, Inc., Englewood Cliffs, N. J. (1962).
Lines, P., Power Numbers for Pitched Blade Turbines. ICI PLC. Report No. (1989).
McCabe, W.L. and Smith, J.C., Unit Operations of Chemical Engineering, 3 ed. McGraw Hill Book Co., New York, N. Y. (1976).
Pandya, J.D. and Spielman, L.A., Floc Breakage in Agitated Suspensions: Theory and Data Processing Strategy. J. Colloid Interface Sci. 90: 517-531 (1982).
Rice, S.A. and Harris, F.E., A Chain Model for Polyelectrolytes II. J. Phys. Chem. 58: 733-739 (1954).
Saffman, P.G. and Turner, J.S., On the Collision of Drops in Turbulent Clouds. J. Fluid Mech. 1: 16 (1956).
Sajjad, M.W. and Cleasby, J.L., Effect of Mixing on Flocculation Kinetics of Kaolin Clay Suspension at Cold Temperature Using Fe(III) Coagulant. pp. 55-62. In: National Conference on Innovative Technologies for Site Remediation and Hazardous Waste Management, Pittsburgh, PA (1995).
Smoluchowski, M. v., Versuch einer Mathematischen Theorie der Koagulationskinetik Kolloide Losungen. Zeitschr. Phys. Chem. 92: 129 (1917).
van de Ven, T.G.M. and Mason, S.G., Comparison of Hydrodynamic and Colloidal Forces in Paper Machine Headboxes. Tappi. 64(9): 171-175 (1981).
van de Ven, T.M.G. and Mason, S.G., The Microrheology of Colloidal IV. Pairs of Interacting Spheres in Shear Flow. J. Colloid Interface Sci. 57: 505 (1976).
van de Ven, T.M.G. and Mason, S.G., The Microrheology of Colloidal VII. Orthokinetic Doublet Formation of Spheres. Colloid and Polymer Sci.
255: 468-479 (1977).
**
To whom correspondence should be addressed.
- Amirtharajah, A., Initial Mixing. pp. 1-22. In: AWWA Seminar on Coagulation and Filtration: Back to the Basics, St. Louis, MO (1981).
- Bradford, M.M., A Rapid and Sensitive Method for the Quantitation of Microgram Quantities of Protein Utilizing the Principle of Protein-Dye Binding. Analyt. Biochem. 72: 248-254 (1976).
- Camp, T.R. and Stein, P.C., Velocity Gradients and Internal Work in Fluid Motion. J. Boston Soc. Civ. Engrs. 30: 219 (1943).
- Carbonell, R.G.; Chen, L.A. and Serad, G.A., Methods of Treating Wastewater. U.S. Patent No. 5,695,647 (1997).
- Chang, Y.H.; Sheldon, B.W. and Carawan, R.E., Research Note: Reconditioning of Prechiller Overflow Water Using a Diatomaceous Earth Pressure Leaf Filter. Poultry Sci. 68: 447-449 (1989).
- Chen, L.A., Recovery of Proteins and Other Biological Compounds from Model and Actual Systems Using Fibrous Materials. Ph.D. Dissertation, North Carolina State University, Raleigh, NC (1996).
- Chen, L.A., Serad, G.A. and Carbonell, R.G., Protein Recovery Using Fibrous Materials, pp. 83-98. In: Butterfield, D. A. (ed.), Biofunctional Membranes. Plenum Press, New York (1996).
- Chen, W. and Berg, J.C., The Effect of Polyelectrolyte Dosage on Floc Formation in Protein Precipitation by Polyelectrolytes. Chem. Eng. Sci. 48(10): 1775-1784 (1993).
- EPA, Suspended Solids Removal. Process Design Manual. Environmental Protection Agency, Washington, D.C. (1975).
- Fisher, R.R. and Glatz, C.E., Polyelectrolyte Precipitation of Proteins: I. The Effects of Reactor Conditions. Biotech. Bioeng. 32: 777-785 (1987).
- François, R.J., Strength of Aluminium Hydroxide Flocs. Water Research. 21(9): 1023-1030 (1987).
- Glasgow, L.A. and Luecke, R.H., Mechanisms of Deaggregation for Clay-Polymer Flocs in Turbulent Systems. Ind. Eng. Chem. Fund. 19: 148-156 (1980).
- Gregory, J., Kinetic Aspects of Polymer Adsorption and Flocculation, pp. 31-45. In: Attia, Y. A. (ed.), Flocculation in Biotechnology and Separation Systems. Elsevier Science Publishers B. V., Amsterdam (1987).
- Hiemenz, P.C., Principles of Colloid and Surface Chemistry, 2d ed. Dekker, New York (1986).
- Ives, K.J., Rate Theories, pp. 39-61. In: Ives, K. J. (ed.), The Scientific Basis of Flocculation. Sijthoff & Noordhoff, Alphen aan den Rijn - The Netherlands (1978).
- Kolmogoroff, A.N., The Local Structure of Turbulence in Incompressible Viscous Fluid for Very Large Reynolds Numbers. C. R. Acad. Sci. U. S. S. R. 30: 301 (1941).
- Kolmogoroff, A.N., On Degeneration of Isotropic Turbulence in an Incompressible Viscous Liquid. C. R. Acad. Sci. U. S. S. R. 31: 538 (1941).
- Letterman, R.D., Flocculation. pp. 45-57. In: AWWA Seminar on Coagulation and Filtration: Back to the Basics, St. Louis, MO (1981).
- Leu, R.-J. and Ghosh, M.M., Polyelectrolyte Characteristics and Flocculation. J. AWWA. 80(4): 159-167 (1988).
- Levich, V.G., Physicochemical Hydrodynamics, 2d ed. Prentice-Hall, Inc., Englewood Cliffs, N. J. (1962).
- Lines, P., Power Numbers for Pitched Blade Turbines. ICI PLC. Report No. (1989).
- McCabe, W.L. and Smith, J.C., Unit Operations of Chemical Engineering, 3 ed. McGraw Hill Book Co., New York, N. Y. (1976).
- Pandya, J.D. and Spielman, L.A., Floc Breakage in Agitated Suspensions: Theory and Data Processing Strategy. J. Colloid Interface Sci. 90: 517-531 (1982).
- Rice, S.A. and Harris, F.E., A Chain Model for Polyelectrolytes II. J. Phys. Chem. 58: 733-739 (1954).
- Saffman, P.G. and Turner, J.S., On the Collision of Drops in Turbulent Clouds. J. Fluid Mech. 1: 16 (1956).
- Sajjad, M.W. and Cleasby, J.L., Effect of Mixing on Flocculation Kinetics of Kaolin Clay Suspension at Cold Temperature Using Fe(III) Coagulant. pp. 55-62. In: National Conference on Innovative Technologies for Site Remediation and Hazardous Waste Management, Pittsburgh, PA (1995).
- Smoluchowski, M. v., Versuch einer Mathematischen Theorie der Koagulationskinetik Kolloide Losungen. Zeitschr. Phys. Chem. 92: 129 (1917).
- van de Ven, T.G.M. and Mason, S.G., Comparison of Hydrodynamic and Colloidal Forces in Paper Machine Headboxes. Tappi. 64(9): 171-175 (1981).
- van de Ven, T.M.G. and Mason, S.G., The Microrheology of Colloidal IV. Pairs of Interacting Spheres in Shear Flow. J. Colloid Interface Sci. 57: 505 (1976).
- van de Ven, T.M.G. and Mason, S.G., The Microrheology of Colloidal VII. Orthokinetic Doublet Formation of Spheres. Colloid and Polymer Sci. 255: 468-479 (1977).
Publication Dates
-
Publication in this collection
07 Dec 1998 -
Date of issue
Dec 1998
History
-
Accepted
01 Oct 1998 -
Received
09 Mar 1998